Authors: Scoffoni C1, Sack L2
Reviewer: Dickman LT3, Johnson DM4
Measurement unit: Kleaf in mmol m-2 s-1 MPa-1, and P50 in MPa; Measurement scale: leaf; Equipment costs: €€€; Running costs: €; Installation effort: low; Maintenance effort: low; Knowledge need: medium; Measurement mode: data logger
A leaf hydraulic vulnerability curve represents the response of the leaf hydraulic conductance (Kleaf – a measure of how efficiently water can move through the leaf) to increasing dehydration for a given species. The leaf hydraulic vulnerability curve is typically depicted by plotting Kleaf against leaf water potential (Ψleaf) (Figure 5.16.1). Because water moves through both the vein xylem and living cells outside the xylem, during soil or atmospheric drought, several phenomena can lead to Kleaf decline: embolism of xylem conduits (i.e. they become filled with air, decreasing the conductance through the xylem; Johnson et al., 2012; Brodribb et al., 2016b), xylem wall collapse (Cochard et al., 2004; Zhang et al., 2016), or cell volume changes (Scoffoni et al., 2014) which are associated with reduced aquaporin activity (Kim & Steudle, 2007; Shatil-Cohen et al., 2011; Sade et al., 2014). While the decline in Kleaf with
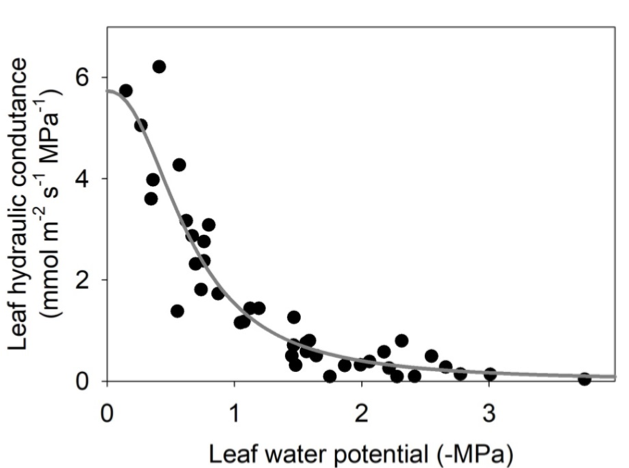
dehydration has most typically been associated with xylem embolism (Scoffoni & Sack, 2017), recent work using x-ray microcomputed tomography and novel methods to partition the xylem and outside-xylem vulnerabilities shows that xylem embolism typically occurs only with very strong dehydration, and changes in outside-xylem pathways can play a major role in Kleaf decline (Trifilo et al., 2016; Scoffoni et al., 2017a). Several functional traits can be extrapolated from the leaf hydraulic vulnerability curve: the leaf water potential at which Kleaf declined by 50% (P50) or 80% (P80), the initial slope of the leaf vulnerability curve, and the maximum leaf hydraulic conductance (Scoffoni et al., 2012). These traits vary widely across species and growth forms and are importantly linked with plant adaptation across biomes (Scoffoni & Sack, 2017). They can shed light on expected responses to more frequent occurrences of drought episodes projected for many regions around the world.
5.16.1 What and how to measure?
There are three methods currently in use to construct leaf hydraulic vulnerability curves: the evaporative flux method, the rehydration method, and the vacuum pump method. The evaporative flux method (EFM) has the advantage of mimicking the natural pathways of water movement in the leaf, including the outside-xylem pathways and therefore is the only method described here. Measuring Kleaf for dehydrated leaves is similar to measuring Kleaf for fully hydrated leaves as described in protocol 5.10 Maximum leaf hydraulic conductance: the main difference is that branches are dehydrated prior to measurement. Kleaf is calculated by dividing flow rate by the leaf water potential at the end of the measurement, but is typically plotted against the most negative water potential it experienced (whether initially after dehydration prior to measurement, or at the end of the measurement if it dehydrated further on the system) to construct leaf hydraulic vulnerability curves. It can take 2–10 days to construct a vulnerability curve for a given species, depending on the number of measurement systems, how vulnerable the species is, and how variable Kleaf is within a given range of water potential. Step-by-step protocols and videos are available (Sack & Scoffoni, 2012).
Branches can be collected in the field the day prior to measurements and placed in large dark plastic bags filled with wet paper towels. Plant material can be transported to the lab in a cooler. In the lab, at least two nodes should be cut under water to ensure the removal of any embolized conduits (as a result of cutting shoots off individuals in air in the field), and the shoots should be left to rehydrate in pure water overnight, with two dark plastic bags filled with wet paper towels covering them, ensuring high atmospheric humidity around the rehydrating samples. Alternatively, if working with greenhouse plants, whole pots can be transported to the lab the evening prior to measurements, watered to saturation and covered in two dark plastic bags filled with wet paper towers. The next day, branches of at least three leaves are placed to dehydrate on the bench (or over a fan) for different amounts of time to obtain a range of initial leaf water potentials (Ψinitial). The branch is then bagged and left to equilibrate for 30 min. Initial water potentials are measured for the top and bottom leaf of the branch. If the two leaves differ by > 0.2 MPa, the branch is discarded. The remaining middle leaf is then attached to the EFM apparatus and Kleaf is measured following the instructions from protocol 5.10 Maximum leaf hydraulic conductance. At the end of the measurement, leaf temperature is recorded, and the leaf taken off the system and its petiole dabbed dry. The leaf is quikly placed in a bag to equilibrate for 30 min before the final leaf water potential is measured (Ψfinal). Kleaf is calculated by dividing the steady-state flow rate (normalised by leaf area and temperature to correct for water viscosity) by Ψfinal. To construct the leaf hydraulic vulnerability curve, Kleaf is plotted against the most negative water potential it experienced (either Ψinitial or Ψfinal). Generally, 6 points per 0.5 MPa intervals are recommended to obtain a species’ vulnerability curve (although the interval can be reduced if the species is very drought sensitive, and the number of points within intervals can be increased if the variation within that interval is too large). Because both linear and non-linear responses of Kleaf to dehydration can be found across species (Brodribb & Holbrook, 2006; Scoffoni et al., 2012), we recommend using a maximum likelihood approach to select and parameterise the best fitting response from at least four functions (linear, sigmoidal, logistic, exponential), all of which have been used in past studies (Scoffoni et al., 2012). From the selected model, the water potential at 50 and 80% loss of Kleaf, as well as the initial slope, can be extrapolated.
Where to start
Guyot et al. (2012), Sack & Scoffoni (2012), Scoffoni & Sack (2017), Scoffoni et al. (2012, 2017a)
5.16.2 Special cases, emerging issues, and challenges
It is imperative to construct vulnerability curves using high light acclimated leaves. This is easily (and typically) done with the EFM and vacuum pump method by placing a bright light source above the leaf (Sack et al., 2002). Studies have shown a strong interaction of the light enhancement of Kleaf with the dehydration response, with species typically showing greater vulnerability in their Kleaf responses under high light between full turgor and turgor loss point (Guyot et al., 2012). Notably, studies using the rehydration kinetics method are typically performed under lab light conditions (e.g. Blackman et al., 2010; Johnson et al., 2012) or under 50–100 μmol quanta m-2 s-1 for only a few minutes while the leaf is rehydrating (e.g. Brodribb et al., 2016b), which could greatly influence the shape and intensity of the response of Kleaf to dehydration. Thus caution is needed when comparing the rehydration kinetics method to the EFM. While a study found the two methods produce comparable results in olive and almond (Hernandez-Santana et al., 2016), not all species respond to light at the same intensity, and further tests are needed to compare these two methods.
One emerging issue has been linked to the interpretation of the causes behind the decline observed in Kleaf. A recent meta-analysis of the literature revealed that the bulk of the species studied (> 300) decline by more than 50% prior to turgor loss point (Scoffoni & Sack, 2017). The majority of studies have assumed that the decline in Kleaf relates to embolism in the vein xylem, as has been widely demonstrated for stems. However, on average across species, 57% of the resistance of the hydraulic pathway is located outside the vein xylem (Scoffoni & Sack, 2017). Recent studies imaging embolism formation in the veins of dehydrated leaves (using either a simple scanner or x-ray microcomputer tomography) reveal that embolism only occurs in strongly dehydrated leaves, typically past their wilting point when stomata are shut (Brodribb et al., 2016a; Hochberg et al., 2017; Scoffoni et al., 2017b), and hydraulics experiments show that strong declines of Kleaf are driven by changes in outside-xylem pathways (Trifilo et al., 2016; Scoffoni et al., 2017a). However, because many processes occur outside the xylem during leaf dehydration (such as aquaporin deactivation, tissue shrinkage, abscisic acid (ABA) signalling, changes in the volume of intercellular airspaces, collapse of xylem conduits in the minor veins), it has been challenging to determine the main cause, although spatially explicit modelling of the outside-xylem pathways have given important insights, pointing especially to a role of declining membrane permeability (Buckley et al., 2017; Scoffoni et al., 2017a).
5.16.3 References
Theory, significance, and large datasets
Blackman et al. (2010), Brodribb et al. (2016a), Hernandez-Santana et al. (2016), Scoffoni & Sack (2017), Trifilo et al. (2016)
More on methods and existing protocols
Guyot et al. (2012), Sack & Scoffoni (2012), Scoffoni et al. (2012)
All references
Blackman, C. J., Brodribb, T. J., & Jordan, G. J. (2010). Leaf hydraulic vulnerability is related to conduit dimensions and drought resistance across a diverse range of woody angiosperms. New Phytologist, 188(4), 1113-1123.
Brodribb, T. J., & Holbrook, N. M. (2006). Declining hydraulic efficiency as transpiring leaves desiccate: two types of response. Plant, Cell & Environment, 29(12), 2205-2215.
Brodribb, T. J., Bienaime, D., & Marmottant, P. (2016a). Revealing catastrophic failure of leaf networks under stress. Proceedings of the National Academy of Sciences USA, 113(17), 4865-4869.
Brodribb, T. J., Skelton, R. P., McAdam, S. A. M., Bienaime, D., Lucani, C. J., & Marmottant, P. (2016b). Visual quantification of embolism reveals leaf vulnerability to hydraulic failure. New Phytologist, 209(4), 1403-1409.
Buckley, T. N., John, G. P., Scoffoni, C., & Sack, L. (2017). The sites of evaporation within leaves. Plant Physiology, 173, 1763-1782.
Cochard, H., Froux, F., Mayr, F. F. S., & Coutand, C. (2004). Xylem wall collapse in water-stressed pine needles. Plant Physiology, 134(1), 401-408.
Guyot, G., Scoffoni, C., & Sack, L. (2012). Combined impacts of irradiance and dehydration on leaf hydraulic conductance: insights into vulnerability and stomatal control. Plant, Cell & Environment, 35, 857-871.
Hernandez-Santana, V., Rodriguez-Dominguez, C. M., Fernández, J. E., & Diaz-Espejo, A. (2016). Role of leaf hydraulic conductance in the regulation of stomatal conductance in almond and olive in response to water stress. Tree Physiology, 36(6), 725-735.
Hochberg, U., Windt, C. W., Ponomarenko, A., Zhang, Y.-J., Gersony, J., Rockwell, F. E., & Holbrook, N. M. (2017). Stomatal closure, basal leaf embolism, and shedding protect the hydraulic integrity of grape stems. Plant Physiology, 174(2), 764-775.
Johnson, D. M., McCulloh, K. A., Woodruff, D. R., & Meinzer, F. C. (2012). Evidence for xylem embolism as a primary factor in dehydration-induced declines in leaf hydraulic conductance. Plant, Cell & Environment, 35(4), 760-769.
Kim, Y. X., & Steudle, E. (2007). Light and turgor affect the water permeability (aquaporins) of parenchyma cells in the midrib of leaves of Zea mays. Journal of Experimental Botany, 58(15-16), 4119-4129.
Sack, L., & Scoffoni, C. (2012). Measurement of leaf hydraulic conductance and stomatal conductance and their responses to irradiance and dehydration using the evaporative flux methods (EFM). Journal of Visualized Experiments, 70, e4179.
Sack, L., Melcher, P. J., Zwieniecki, M. A., & Holbrook, N. M. (2002). The hydraulic conductance of the angiosperm leaf lamina: a comparison of three measurement methods. Journal of Experimental Botany, 53(378), 2177-2184.
Sade, N., Shatil-Cohen, A., Attia, Z., Maurel, C., Boursiac, Y., Kelly, G., … Moshelion, M. (2014). The role of plasma membrane aquaporins in regulating the bundle sheath-mesophyll continuum and leaf hydraulics. Plant Physiology, 166(3), 1609-1620.
Scoffoni, C., & Sack, L. (2017). The causes and consequences of leaf hydraulic decline with dehydration. Journal of Experimental Botany, 68(16), 4479-4496.
Scoffoni, C., McKown, A. D., Rawls, M., & Sack, L. (2012). Dynamics of leaf hydraulic conductance with water status: quantification and analysis of species differences under steady-state. Journal of Experimental Botany, 63, 643-658.
Scoffoni, C., Vuong, C., Diep, S., Cochard, H., & Sack, L. (2014). Leaf shrinkage with dehydration: coordination with hydraulic vulnerability and drought tolerance. Plant Physiology, 164, 1772-1788.
Scoffoni, C., Albuquerque, C., Brodersen, C. R., Townes, S. T., John, G. P., Bartlett, M. K., … Sack, L. (2017a). Outside-xylem tissue vulnerability, not xylem embolism, controls leaf hydraulic decline with dehydration across diverse angiosperms. Plant Physiology, 173, 1197-1210.
Scoffoni, C., Albuquerque, C., Brodersen, C. R., Townes, S. T., John, G. P., Cochard, H., … Sack, L. (2017b). Leaf vein xylem conduit diameter influences susceptibility to embolism and hydraulic decline. New Phytologist, 213, 1076-1092.
Shatil-Cohen, A., Attia, Z., & Moshelion, M. (2011). Bundle-sheath cell regulation of xylem-mesophyll water transport via aquaporins under drought stress: a target of xylem-borne ABA? Plant Journal, 67(1), 72-80.
Trifilo, P., Raimondo, F., Savi, T., Lo Gullo, M. A., & Nardini, A. (2016). The contribution of vascular and extra-vascular water pathways to drought-induced decline of leaf hydraulic conductance. Journal of Experimental Botany, 67(17), 5029-5039.
Zhang, Y. J., Rockwell, F. E., Graham, A. C., Alexander, T., & Holbrook, N. M. (2016). Reversible leaf xylem collapse: a potential “circuit breaker” against cavitation. Plant Physiology, 172(4), 2261-2274.
Authors: Scoffoni C1, Sack L2
Reviewer: Dickman LT3, Johnson DM4
Affiliations
1 Department of Biological Sciences, California State University, Los Angeles, USA
2 Department of Ecology and Evolutionary Biology, University of California Los Angeles, Los Angeles, USA
3 Earth and Environmental Sciences Division, Los Alamos National Laboratory, Los Alamos, USA
4 Warnell School of Forestry and Natural Resources, University of Georgia, Athens, USA